by SPIE
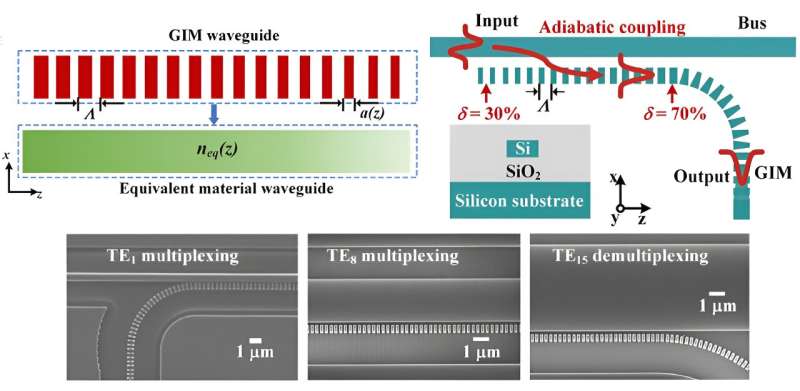
The past few years have seen a massive surge in the amount of data transferred and processed per second. Rapidly emerging technologies, such as high-dimensional quantum communications, large-scale neural networks, and high-capacity networks, require large bandwidths and high data transfer speeds. One plausible way to achieve them is by replacing the conventional metallic wires between the components in an electronic system with optical interconnects, i.e., using light instead of electricity to establish channels for data transfer.
Optical interconnections can provide incredibly high speeds via a technique known as mode-division multiplexing (MDM). Thanks to precisely designed structures called waveguides, light can propagate in specific patterns called “modes.” Since multiple modes can propagate in the same medium simultaneously without interfering with each other, they effectively act as separate data channels, increasing the overall data transfer rate of the system.
However, the speed of MDM systems reported so far has been limited, mainly due to the imperfections in the device fabrication that cause the refractive index variations of the waveguides. One way to mitigate the imperfections is to carefully engineer the refractive indices of the waveguides by optimizing the structure and composition. Unfortunately, currently available methods are limited by either the choice of materials or the resulting large circuit footprint.
Against this backdrop, a research team including Professor Yikai Su from Shanghai Jiao Tong University in China sought to develop a new approach for coupling (or combining) different light modes. As reported in their study published in Advanced Photonics, the team successfully employed this technique in an MDM system, achieving unprecedented data rates.
The main highlight of the research is an innovative design for a light-mode coupler, a structure that can manipulate a specific light mode traveling in a nearby bus waveguide, such as a nanowire carrying the total multi-mode signal. The coupler can inject a desired light mode into the bus waveguide or extract one from it, sending it towards a different path.
The researchers tailored the refractive index of the coupler such that it interacted strongly with the desired light mode in a wide range of coupling region in the presence of fabrication errors, thus realizing a high coupling coefficient. They achieved this by leveraging a gradient-index metamaterial (GIM) waveguide.
Contrary to usual materials, the GIM exhibited a refractive index that varied continuously along the direction of propagation of light. This, in turn, facilitated a seamless and efficient transition of individual light modes to and from the nanowire bus by mitigating the parameter variations of the waveguides.
By cascading multiple couplers, the researchers created a 16-channel MDM communication system that supported 16 different light modes—TE0 to TE15—simultaneously. In a data transmission experiment, it achieved a data transfer rate of 2.162 Tbit/s, the highest ever reported value for an on-chip device operating at a single wavelength.
Moreover, the system was fabricated using methods that are compatible in semiconductor device fabrication, such as electron beam lithography, plasma etching, and chemical vapor deposition. This made the design easily scalable and compatible with currently available fabrication technology.
Overall, the proposed coupling strategy using a GIM structure may provide a much-needed boost in data rates, especially in fields where large-scale parallel data transmissions and computations are common. This could translate to new benchmarks in hardware acceleration, large-scale neural networks, and quantum communications.
More information: Yu He et al, On-chip metamaterial-enabled high-order mode-division multiplexing, Advanced Photonics (2023). DOI: 10.1117/1.AP.5.5.056008
Journal information: Advanced Photonics
Provided by SPIE