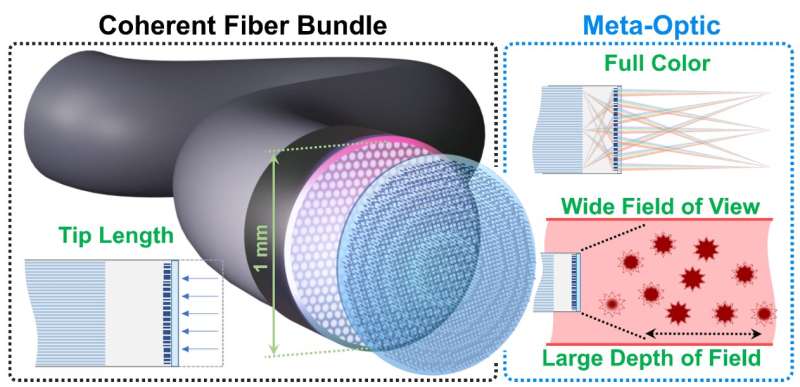
Ultra-compact, agile endoscopes with a large field of view (FoV), long depth of field (DoF), and short rigid tip length are essential for developing minimally invasive operations and new experimental surgeries. As these fields develop, the requirement for miniaturization and increased precision become progressively demanding.
In existing endoscopes, the rigid tip length is a fundamental limitation of the device’s agility within small tortuous ducts, such as an artery. It is primarily constrained by the size of the optical elements required for imaging. Thus, alternative solutions are urgently needed to reduce the tip length.
In a new paper published in eLight, a team of scientists led by Dr. Johannes Fröch and Prof Arka Majumdar from the University of Washington have developed a novel technique for reducing the rigid tip length.
Existing solutions include lensless and computational imaging with single fibers or coherent fiber bundles. However, these are typically limited to a short working distance and often extremely sensitive to bending and twisting of the optical fiber, affecting or even precluding accurate computational reconstruction.
Flat meta-optics are an emerging and versatile idea in the photonics community to create miniaturized optical elements. These are sub-wavelength diffractive optical elements composed of nano-scale scatterer arrays. They are designed to shape an incident wavefront’s phase, amplitude, and spectral response. Such ultrathin flat optics not only dramatically shrink the size of traditional optics but can also combine multiple functionalities in a single surface.
Flat meta-optics are compatible with high-volume semiconductor manufacturing technology and can create disposable optics. These properties have already inspired researchers to explore the potential of meta-optics for endoscopy, including fiber-integrated endoscopy, side-viewing single fiber scanning endoscopy, and scanning fiber forward-viewing endoscopy.
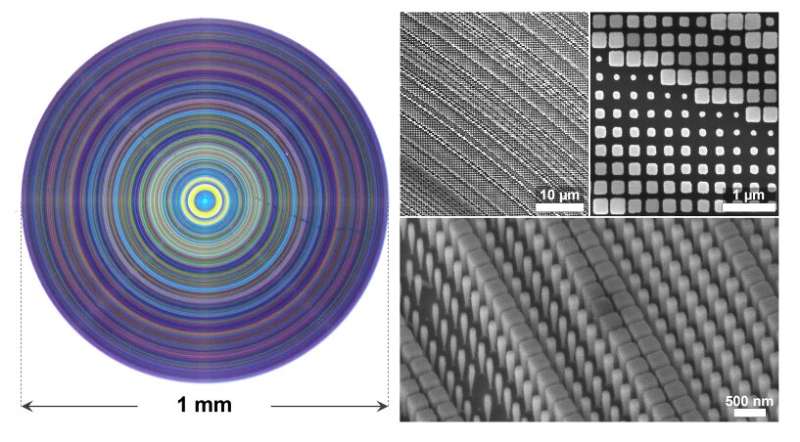
Unfortunately, meta-optics traditionally suffer from strong aberrations, making large FoV and full-color imaging challenging. Several works have shown that the standard metalens design is unsuitable for simultaneously capturing color information across the visible spectrum.
It typically results in crisp images for the design wavelength (e.g. green) but strongly aberrated/ blurred for other colors (red and blue). While some approaches like dispersion engineering and computational imaging techniques can reduce chromatic aberration, they either suffer from small apertures, low numerical apertures or require a computational post-processing step, complicating real-time video capture.
Similarly, an additional aperture before the meta-optic can provide a larger FoV. However, it comes at the cost of reduced light collection and increased thickness of the optics. So far, these limitations have restricted most meta-optics endoscopes to single wavelength operation.
Although, recently, a meta-optic doublet was demonstrated in conjunction with a coherent fiber bundle for polychromatic imaging. Such polychromatic imaging is unsuitable for broad-band illumination, which is often the case for clinical endoscopy. Additionally, the front aperture was limited to 125 μm, with a short working distance of 200 μm.
The research team noted a desire for broad-band, ultra-thin meta-optics for endoscopy. However, making it smaller than the optical fiber diameter is not conducive and severely limits the light collection. As such, full-color meta-optical endoscopy with acceptable FoV, DoF, and large enough aperture has not yet been achieved.
In this work, the research team demonstrated an inverse-designed meta-optic optimized to capture real-time full color scenes with a 1 mm diameter coherent fiber bundle. The meta-optic enables operations at an FoV of 22.5°, a DoF of > 30 mm (exceeding 300% of the nominal design working distance) and a minimum rigid tip length of only ~ 2.5 mm.
This is a 33% tip length reduction compared to a traditional commercial gradient-index (GRIN) lens integrated fiber bundle endoscope. This is due to the shorter focal length and the ultrathin nature of the meta-optic.
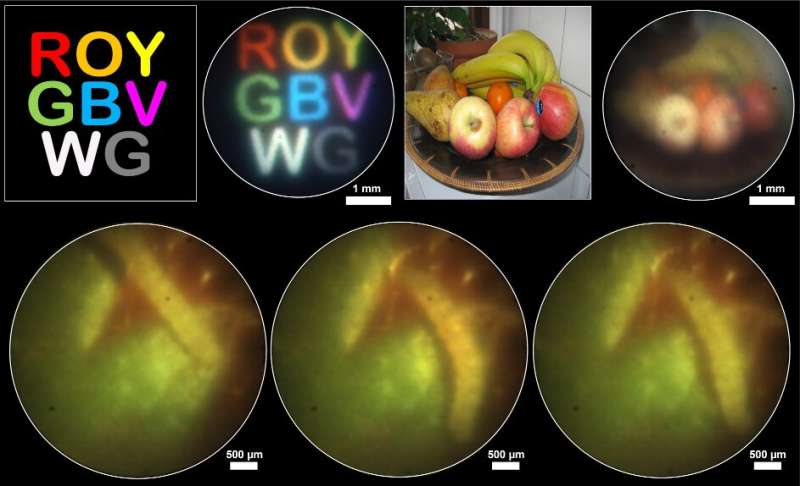
At the same time, comparable imaging performance and working distance are maintained. To achieve exceptional FoV, DoF, and color performance of the Meta-Optical Fiber Endoscope (MOFIE), the research team approached this design problem from a system-level perspective.
They believed that the diameter and spacing of individual fiber cores within the bundle limit the achievable image quality, which also limits the achievable FoV and modulation transfer function (MTF). This aspect is implemented in an automatic differentiation framework using the average volume under the multichromatic modulation transfer function (MTF) curve as the figure of merit.
By ensuring that the meta-optic has an MTF within the limitations of the fiber bundle, the research team achieved full-color operation without requiring a computational reconstruction step, thus facilitating real-time operation. The team emphasized that its design approach fundamentally differs from traditional achromatic metalens design efforts.
The researchers formulated an optimization problem to find the best solution for full-color imaging. This was instead of trying to achieve diffraction-limited performance in all wavelengths, which may pose a physically unsolvable problem.
This approach is important because it is not limited to this particular system. It can be extended to larger aperture sizes and support computational post-processing steps. To highlight this, they also demonstrated an example of a meta-optic with a 1 cm aperture and full-color imaging under ambient light conditions.
More information: Johannes E. Fröch et al, Real time full-color imaging in a Meta-optical fiber endoscope, eLight (2023). DOI: 10.1186/s43593-023-00044-4
Provided by Chinese Academy of Sciences