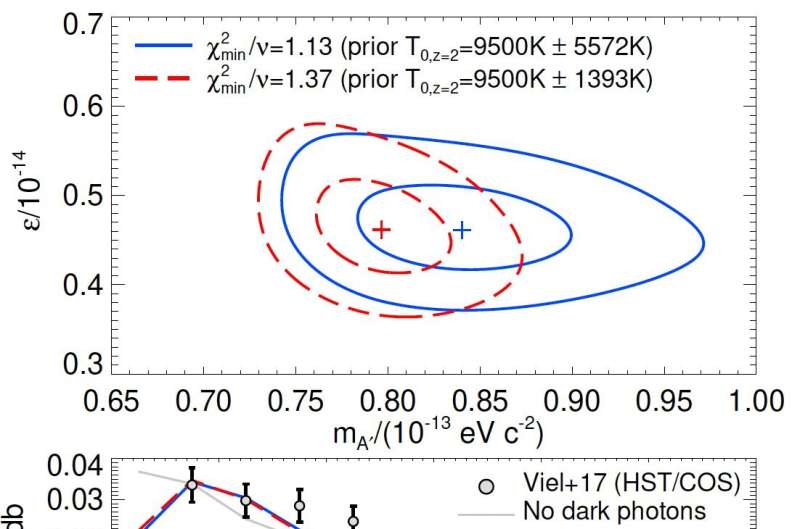
Gas clouds across the universe are known to absorb the light produced by distant massive celestial objects, known as quasars. This light manifests as the so-called Lyman alpha forest, a dense structure composed of absorption lines that can be observed using spectroscopy tools.
Over the past decades, astrophysicists have been assessing the value of these absorption lines as a tool to better understand the universe and the relationships between cosmological objects. The Lyman alpha forest could also potentially aid the ongoing search for dark matter, offering an additional tool to test theoretical predictions and models.
Researchers at University of Nottingham, Tel-Aviv University, New York University, and the Institute for Fundamental Physics of the Universe in Trieste have recently compared low-redshift Lyman alpha forest observations to hydrodynamical simulations of the intergalactic medium and dark matter made up of dark photons, a renowned dark matter candidate.
Their paper, published in Physical Review Letters (PRL), builds on an earlier work by some members of their team, which compared simulations of the intergalactic medium (IGM) with Lyman-alpha forest measurements collected by the Cosmic Origins Spectrograph (COS) aboard the Hubble Space Telescope.
“In our analyses, we found that the simulation predicted line widths that were too narrow compared to the COS results, suggesting that there could be additional, noncanonical sources of heating occurring at low redshifts,” Hongwan Liu, Matteo Viel, Andrea Caputo and James Bolton, the researchers who carried out the study, told Phys.org via email.
“We explored several dark matter models that could act as this source of heating. Building on two of the authors’ experience with dark photons in a previous paper published in PRL, we eventually realized that heating from dark photon dark matter could work.”
Based on their previous observations, Liu, Viel, Caputo and Bolton decided to alter a hydrodynamical simulation of the IGM (i.e., a sparse cloud of hydrogen that exists in the spaces between galaxies). In their new simulation, they included the effects of the heat that models predict would be produced by dark photon dark matter.
“In regions of space where the mass of the dark photon matches the effective plasma mass of the photon, conversions from dark photons to photons can occur,” Liu, Viel, Caputo and Bolton explained. “The converted photons are then rapidly absorbed by the IGM in those regions, heating the gas up. The amount of energy transferred from dark matter to the gas can be calculated theoretically.”
The researchers added this estimated energy transfer between dark photons and intergalactic clouds to their simulations. This ultimately allowed them to attain a series of simulated absorption line widths, which they could compare to actual Lyman-alpha forest observations collected by the COS.
“Broadly speaking, we have shown that the Lyman-alpha forest is extremely useful for understanding dark matter models where energy can be converted from dark matter into heating,” Liu, Viel, Caputo and Bolton said. “I think our study will encourage physicists interested in dark matter to pay more attention to the Lyman-alpha forest.”
Overall, the comparison between COS measurements and hydrodynamical simulations performed by this team of researchers suggests that dark photons could in fact be a source of heat in intergalactic gas clouds. Their findings could thus be the first hint of the existence of dark matter that is not observed through its gravitational effects.
While this is a fascinating possibility, Liu, Viel, Caputo and Bolton have not yet ruled out other possible theoretical explanations. They thus hope that their study will inspire other teams to similarly probe the properties of the IGM in the early universe.
“One particularly interesting consequence of dark photon heating is that underdense regions in the IGM are heated up at earlier times compared to overdense regions,” Liu, Viel, Caputo and Bolton said. “This can lead to underdense regions being hotter than overdense regions, which is contrary to standard expectations. There are some indications that the IGM does exhibit this behavior at high redshifts. If so, it could be another important piece of evidence in favor of dark photon dark matter heating.”
More information: James S. Bolton et al, Comparison of Low-Redshift Lyman- α Forest Observations to Hydrodynamical Simulations with Dark Photon Dark Matter, Physical Review Letters (2022). DOI: 10.1103/PhysRevLett.129.211102
James S Bolton et al, Limits on non-canonical heating and turbulence in the intergalactic medium from the low redshift Lyman α forest, Monthly Notices of the Royal Astronomical Society (2022). DOI: 10.1093/mnras/stac862
Andrea Caputo et al, Dark Photon Oscillations in Our Inhomogeneous Universe, Physical Review Letters (2020). DOI: 10.1103/PhysRevLett.125.221303
Journal information: Monthly Notices of the Royal Astronomical Society , Physical Review Letters