by Luke Auburn, University of Rochester
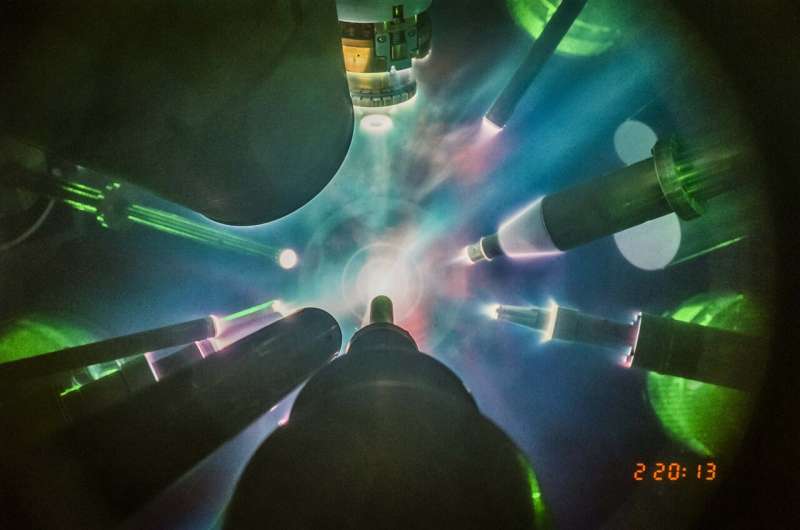
Scientists from the University of Rochester’s Laboratory for Laser Energetics (LLE) led experiments to demonstrate an effective “spark plug” for direct-drive methods of inertial confinement fusion (ICF). In two studies published in Nature Physics, the authors discuss their results and outline how they can be applied at bigger scales with the hopes of eventually producing fusion at a future facility.
LLE is the largest university-based U.S. Department of Energy program and hosts the OMEGA laser system, which is largest academic laser in the world but still almost one hundredth the energy of the National Ignition Facility (NIF) at the Lawrence Livermore National Laboratory in California.
With OMEGA, Rochester scientists completed several successful attempts to fire 28 kilojoules of laser energy at small capsules filled with deuterium and tritium fuel, causing the capsules to implode and produce a plasma hot enough to initiate fusion reactions between the fuel nuclei. The experiments caused fusion reactions that produced more energy than the amount of energy in the central hot plasma.
The OMEGA experiments use direct laser illumination of the capsule and differ from the indirect drive approach used on the NIF. When using the indirect drive approach, the laser light is converted into X-rays that in turn drive the capsule implosion. The NIF used indirect drive to irradiate a capsule with X-rays using about 2,000 kilojoules of laser energy. This led to a 2022 breakthrough at NIF in achieving fusion ignition—a fusion reaction that creates a net gain of energy from the target.
“Generating more fusion energy than the internal energy content of where the fusion takes place is an important threshold,” says lead author of the first paper Connor Williams ’23 Ph.D. (physics and astronomy), now a staff scientist at Sandia National Labs in radiation and ICF target design. “That’s a necessary requirement for anything you want to accomplish later on, such as burning plasmas or achieving ignition.”
By showing they can achieve this level of implosion performance with just 28 kilojoules of laser energy, the Rochester team is excited by the prospect of applying direct-drive methods to lasers with more energy. Demonstrating a spark plug is an important step, however, OMEGA is too small to compress enough fuel to get to ignition.
“If you can eventually create the spark plug and compress fuel, direct drive has a lot of characteristics that are favorable for fusion energy compared to indirect drive,” says Varchas Gopalaswamy ’21 Ph.D. (mechanical engineering), the LLE scientist who led the second study that explores the implications of using the direct-drive approach on megajoule-class lasers, similar to the size of the NIF. “After scaling the OMEGA results to a few megajoules of laser energies, the fusion reactions are predicted to become self-sustaining, a condition called ‘burning plasmas.'”
Gopalaswamy says that direct-drive ICF is a promising approach for achieving thermonuclear ignition and net energy in laser fusion.
“A major factor contributing to the success of these recent experiments is the development of a novel implosion design method based on statistical predictions and validated by machine learning algorithms,” says Riccardo Betti, LLE’s chief scientist and the Robert L. McCrory Professor in the Department of Mechanical Engineering and in the Department of Physics and Astronomy. “These predictive models allow us to narrow the pool of promising candidate designs before carrying out valuable experiments.”
The Rochester experiments required a highly coordinated effort between large number of scientists, engineers, and technical staff to operate the complex laser facility. They collaborated with researchers from the MIT Plasma Science and Fusion Center and General Atomics to conduct the experiments.
More information: C. A. Williams et al, Demonstration of hot-spot fuel gain exceeding unity in direct-drive inertial confinement fusion implosions, Nature Physics (2024). DOI: 10.1038/s41567-023-02363-2
V. Gopalaswamy et al, Demonstration of a hydrodynamically equivalent burning plasma in direct-drive inertial confinement fusion, Nature Physics (2024). DOI: 10.1038/s41567-023-02361-4
Provided by University of Rochester